Key Findings
>> Four human iPSC lines, 3 from donors with ALS and 1 unaffected donor, were successfully differentiated to functional motor neurons.
>> The use of the ‘Accelerator’ supplement to mimic in vivo like environment enabled assay ready, functional firing of motor neurons in ~10 days.
>> Used in conjunction with the Maestro Pro, the ”thaw-to-data” time was ~20 days.
>> Motor neurons derived from ALS donor iPSCs were morphologically different to those from unaffected donors.
>> Through MEA analysis, morphological differences corresponded to functional phenotype differences including the key attribute of hyperexcitability.
Introduction
Amyotrophic Lateral Sclerosis (ALS) is a progressively fatal neurodegenerative disease that damages nerve cells in the central nervous system (CNS), but its effects are manifested in the peripheral nervous system (PNS) due to the loss of motor neuron function resulting in muscle weakness and atrophy. Although ~85-90% of ALS cases are sporadic, mutations in over 40 genes have been identified that contribute to the development of ALS (1) .
Despite considerable research efforts and funding, there is no cure for ALS and approved treatments are primarily aimed at addressing symptoms. A significant hurdle in early-stage drug discovery for ALS is the lack of standardized, easy-to-use, and readily accessible human cell models. As a result, scientists have been constrained to animal models and cell lines that differ considerably from human disease biology, posing a considerable challenge for lead optimization.
One specific trait relating to ALS neuronal pathology is hyperexcitability. Hyperexcitability refers to an increased responsiveness of motor neurons to synaptic inputs, leading to an abnormal increase in firing rate. This altered electrical activity is believed to contribute to the degeneration of motor neurons and the progression of ALS and, as such, should be an essential attribute in an in vitro model.
Human induced pluripotent stem cell (iPSC)-derived disease models, with their direct connection back to a human donor, offer the promise of increased translational relevance when compared to traditional in vitro models. These also allow for a reproducible and scalable supply of cells providing representation of clinically relevant disease phenotypes.
Axol Bioscience is a manufacturing and services company who has worked with neuronal iPSC technology for over a decade. The company aims to develop more effective in vitro models for drug discovery through the production of consistent and functionally relevant cells under ISO 9001 conditions. Cell types include neurons, neuroinflammatory cells, and astrocytes.
Media and cell-specific supplements have been developed to simulate a more “in vivo-like” environment and have been previously demonstrated to accelerate maturation and produce more mature end cell types.
We characterized motor neurons morphologically and functionally, via multielectrode array (MEA), from 4 different lines. Frozen motor neuron progenitors from healthy patients were assay ready from as early as 10 days post-thaw, while SOD1 and TDP-43 phenotypes required 20 days post-thaw to mature (Fig. 1).
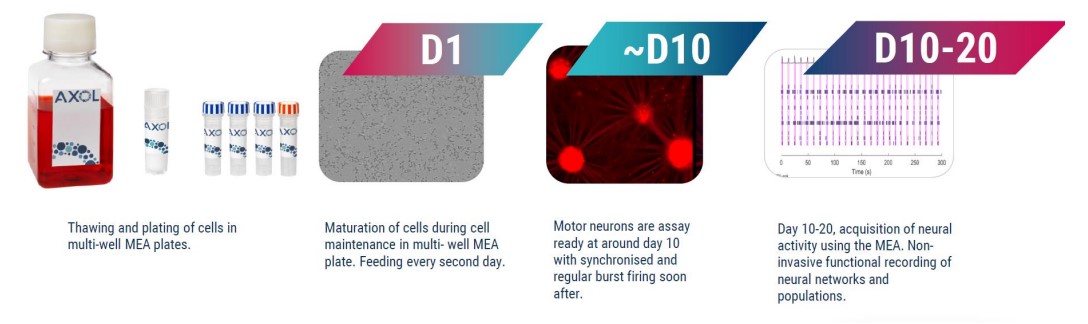
Materials and Methods
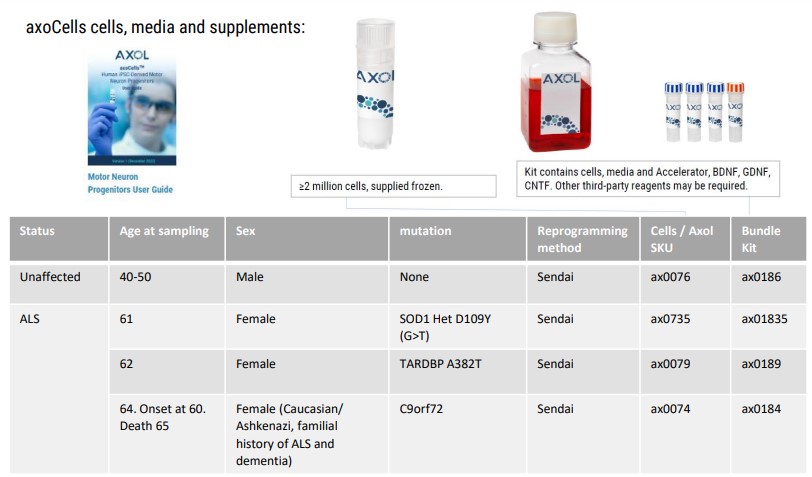
Also used:
- ax0072 – Motor neuron maintenance medium
- ax0178 – Motor neuron Accelerator supplement
Cell plating and measurements
Motor neuron progenitors (Axol Biosciences) were plated at 60K cells per well in a CytoView MEA 48- well plate and cultured for up to 20 days according to manufacturer-recommended protocols (Fig. 2A).
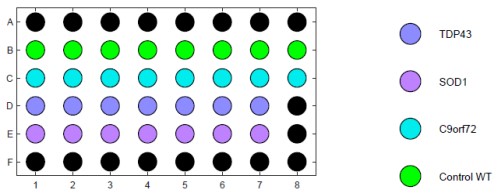
Motor neurons were imaged with brightfield phase microscopy at DIV20; the motor neurons formed a comprehensive monolayer, providing extensive electrode coverage (Fig. 2B).
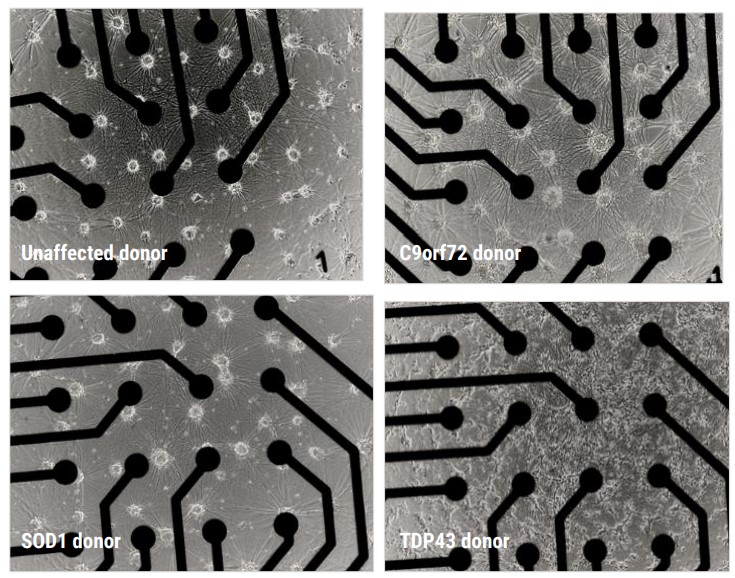
Data was recorded using the “Neural Spontaneous” configuration in AxIS Navigator software (Axion BioSystems) (Fig. 2C).
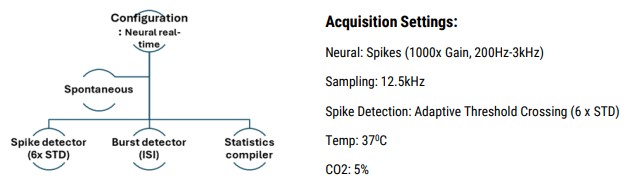
- Data analysis performed using Neural Metric Tool and AxIS Metric Plotting Tool (Axion BioSystems)
- All plotted values exclude wells below 0.1 Hz Mean Firing Rate
Results
Characterization by cell morphology
To verify the cells as suitable disease models for functional screening, it is critical to identify whether the disease models demonstrate a disease relevant phenotype when compared to the control.
The motor neurons derived from unaffected and ALS patients were cultured as per the Axol Bioscience user guides and both their morphology and functional neuronal electrical activity were assessed.
The functional activity of the neurons was measured at several time points on the environmentally controlled Maestro Pro MEA platform. Both unaffected and ALS cells developed a stable monolayer in the plates, with suitable coverage of the electrodes.
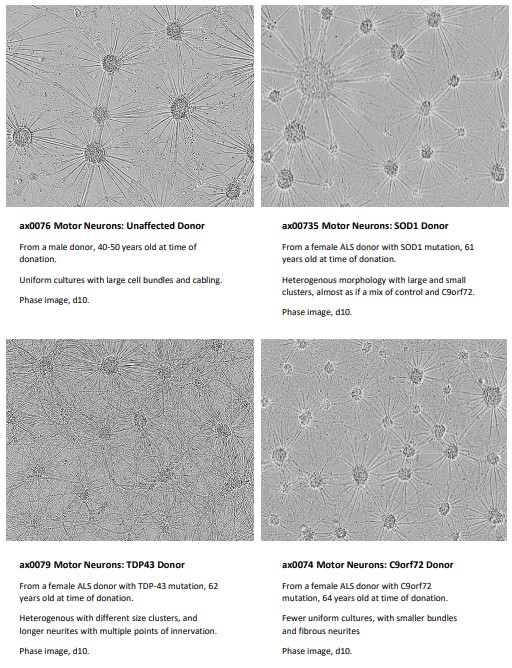
Characterization by MEA
MEA enables non-invasive measurement of electrically active cells within a familiar multi-well cell culture format. MEA measurements are based on the detection of extracellular field potentials, which are the electrical signals generated by the activity of neurons or other excitable cells. These potentials arise due to the flow of ions (such as sodium, potassium, and calcium) across the cell membrane during action potentials or other electrical events.
‘Raw’ data is seen in real-time as a continuous voltage trace. A simple way to visualize this neural network behavior is with a raster plot (Table 2). Each tick mark represents the time, on the x axis, that a spike occurred. Each row of tick marks represents the spikes from a single electrode. Several consecutive spikes make a burst (shown in blue), and when multiple electrodes burst at the same time, this is represented as network burst (pink box).
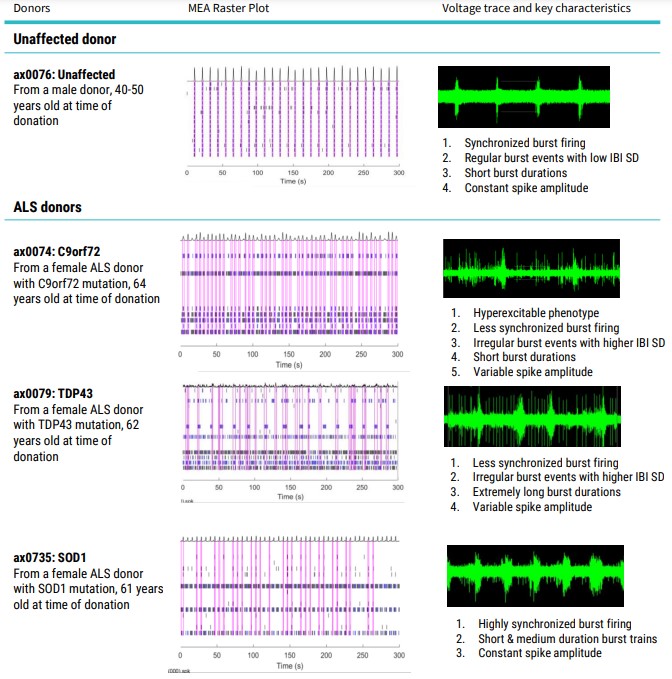
The firing activity of the cells was assessed by 5-minute MEA recordings from DIV 10. Synchronous firing was detected from DIV 8 post-thaw. Data was recorded using the “Neural Spontaneous” configuration in AxIS Navigator software (Axion BioSystems). Action potentials were detected via adaptive thresholding to assess a selection of both non-networked and networked parameters which can be looked at in the module and are outlined in Table 3.
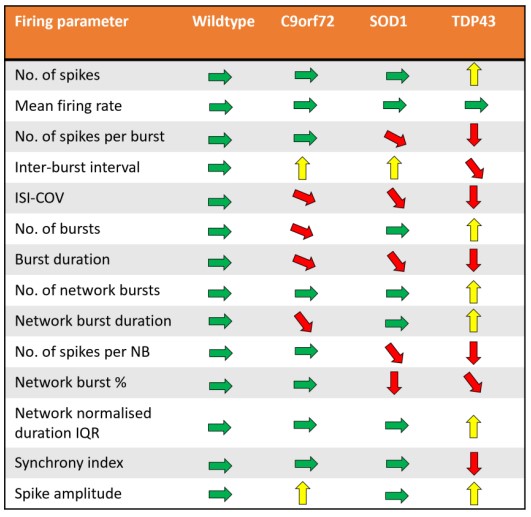
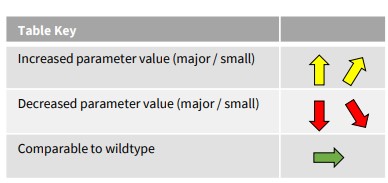
The differing ALS disease lines display clear network firing differences in keeping with their phenotype and disease complexity (Table 2). Samples are from a minimum of 3 electrodes in the same well, demonstrating the differences or uniformity of firing patterns within each cell type.
Quantification of the activity profiles and raster plots for the cultures shows that the ALS motor neurons have distinct firing profiles than the unaffected donor motor neurons. Differences are observed in firing rate, spike amplitude, bursting profiles and networking profiles. All the neurons produce clear burst and network burst activity as seen in the raster plots at DIV 20 (Table 2).
Neural activity recording and analysis with the Maestro MEA platform
Action potentials were detected via adaptive thresholding to be processed and assessed quantitatively as both individual and network parameters, including measurements of firing, bursting, and synchrony. Data analysis was performed using the AxIS Metric Plotting Tool and wells were excluded if below 0.1 Hz Mean Firing Rate to generate the following raster plots (Fig. 3A) and a selection of quantitative endpoints for both non-networked and networked parameters (Fig. 3B). Error bars indicate SEM, n=3 -10 independent technical repeats against 10 wells per condition. Neurons produce clear burst and network burst activity as seen in the raster plots at DIV 20. Table 3 summarizes the graphical data into a comparison table with changes in each listed parameter indicated by color and arrow direction.
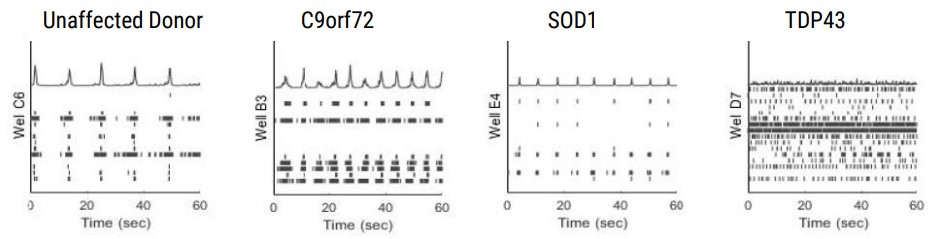
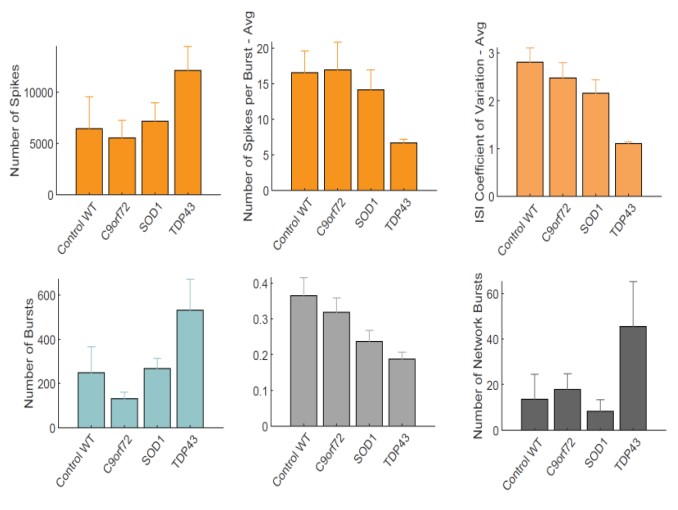
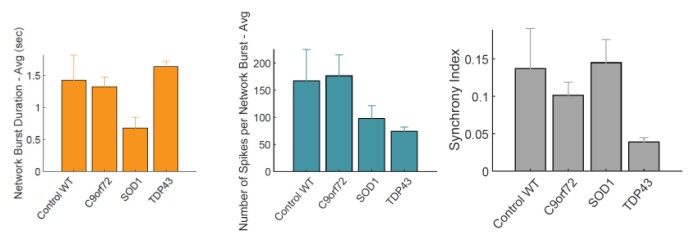
Discussion
In the characterization of this set of axoCellsTM iPSC-derived motor neurons, we used MEA and imaging to observe key traits. By incorporating this characterization into manufacturing QC and functional QC processes, we recorded multiple lines and multiple production runs (differentiations) to ensure these traits were robust and reproducible and were not just a consequence of a single differentiation procedure.
The use of Accelerator supplement and the Maestro Pro MEA enabled a short 20 day “from thaw to data” experimental regime to detect neuronal firing data and enabled simultaneous brightfield imaging for confirmation of cell spotting accuracy and correlation of cell culture health. Motor neurons from 4 different lines were assessed for ALS-related morphology and neural activity phenotypes. The unaffected control line exhibited consistent spike amplitude, short burst events, and synchrony. In comparison, motor neurons from the ALS lines demonstrated a reproducible loss- or gain-of-function for certain parameters in each phenotype. C9orf72 motor neurons displayed increase spike amplitude and shortened ISI with increased network burst frequency. SOD1 motor neurons displayed shortened ISI with decreased network burst % and fewer spikes per burst. TDP-43 motor neurons displayed increased spike amplitude and mean firing rate with highly unsynchronized network activity and bursting patterns.
All ALS phenotype lines displayed a reproducible loss of synchronous firing and different degrees of hyperexcitability. This is in accordance with expected ALS clinical pathology and supports the case for the use of these cells in experimental in vitro models to study ALS pathology and potential therapeutics.